Jonathan Noëla,*, Anya Mascarenhasb, Chibueze A. Nwaiwuc,d, Yao Liuc,d, Marcio Moschovase, Vasiliy E. Buharind, John Oberlind, Saloni Mehrotrad,f, Alyson F. Dechertd, Peter C. W. Kimc,d, Vipul Patela
aDepartment of Urology, AdventHealth Global Robotics Institute, Celebration, FL, USA; bHarvard University, Cambridge, MA, USA; cDepartment of Surgery, Warren Alpert Medical School of Brown University, Rhode Island Hospital, Providence, RI, USA; dDepartment of Research, Activ Surgical Inc., Boston, MA, USA; eDepartment of Urology, AdventHealth Global Robotics Institute, Celebration, FL, USA; fDepartment of Surgery, University of Buffalo, Buffalo, NY, USA
Abstract
Background: When viewed under near-infrared light, indocyanine green (ICG) signal for kidney perfusion can be utilized in partial nephrectomy. Laser speckle contrast imaging (LSCI) uses coherent light to detect perfusion during real-time laparoscopic surgery.
Materials and methods: Laser speckle contrast imaging or ActivSight, an imaging sensor adapter, was used during laparoscopy of an anesthetized porcine kidney model. ActivSight’s “perfusion mode” and “quantification mode” displayed the blood flow as a heatmap and numerical signal intensity, respectively.
Results: After the upper segmental renal artery was clamped, ICG was seen in the lower pole, and LSCI showed low unit (dark color) quantification and perfusion in the upper pole. Indocyanine green was retained in the lower pole after the upper segmental artery was unclamped, and LSCI perfusion was demonstrated in the entire kidney.
Conclusions: Laser speckle contrast imaging is a dye-free, repeatable, real-time adjunct for renal parenchymal perfusion assessment applicable to minimally invasive renal surgery to complement the technology of ICG near-infrared fluorescence and advance digital surgery.
Keywords: Augmented reality; Indocyanine green; Laser speckle contrast imaging; Perfusion; Selective arterial clamping
1. Introduction
Endourology for renal surgery began with Clayman and colleagues’[1] case description of the laparoscopic approach, with embolization of the renal artery immediately before surgery. Laparoscopic nephrectomy is routinely performed for benign and cancerous kidney conditions. The use of partial nephrectomy (PN) has steadily increased,[2] given its equivalence in cancer control compared with radical nephrectomy.[3] Partial nephrectomy can minimize the risk of renal disease progression by sparing nephrons, particularly in patients with comorbidities, single kidneys, or bilateral tumors. However, the increased likelihood of postoperative complications in PN compared with radical nephrectomy[4] has incited the development of intraoperative imaging technologies to improve surgical outcomes.
Among these innovations is the use of fluorescent contrast agents that emit light that can be captured using a high-resolution endoscopic camera. The most utilized and studied fluorescent agent in urologic surgery is indocyanine green (ICG).[5] Indocyanine green is administered intravenously and remains intravascular until it is excreted by the liver. It emits photons with a wavelength of 820 to 830 nm,[6] which are translated to a green color using a near-infrared fluorescence (NIRF) imaging system. The color is visualized in vessels and the renal parenchyma within 1 minute of administration,[7] allowing for selective arterial clamping and tumor localization during PN.
There is a growing body of evidence that ICG fluorescence angiography can readily identify important vasculature. This is useful in donor nephrectomies and kidney transplantation for recipient assessment of perfusion to the allograft, ureteral reconstruction, and complex reconstruction procedures.[8–10] Indocyanine green fluorescence angiography has also been used to identify neurovascular bundles and landmark arteries in nerve-sparing radical prostatectomy. Indocyanine green lymphangiography has also been assessed for sentinel lymph node dissection in radical prostatectomy, cystectomy, and penectomy.[9,11,12] Lastly, ICG may play a role in delineating tumor margins in urological and nonurological radical surgery.[13,14]
Despite these potential benefits, the pharmacokinetics of ICG limits its usability in certain scenarios. For example, ICG-based visualization sometimes requires multiple timed injections of a contrast agent to visualize tissue perfusion. The accuracy of tissue perfusion is reduced by subjective interpretation based on the degree of fluorescence, as well as the likelihood of false-positive information on repeat assessments, because of capillary leakage and dye retention in interstitial tissue.[15–17] Furthermore, the lack of standardized dosage, methodology, and quantifiable measurements increases the chance of error due to subjective interpretation.[16,18]
Laser speckle contrast imaging (LSCI) was developed to decrease the risk of unrecognized ischemia and improve upon ICG using a dye-less, real-time perfusion detection technology. Laser speckle contrast imaging is a technique that uses coherent laser light to detect object movement. The underlying mechanism of LSCI depends on the fact that coherent laser light can interfere with itself. When a target is illuminated by laser light, the image formed is similar to a normal photographic image; however, it has salt-and-pepper “speckles” superimposed upon it. A stationary target has a static field of speckles, and a moving target causes those speckles to twinkle or blink at a rate proportional to the velocity of the underlying target. Thus, when stationary tissue is imaged, the detected movement corresponds to flowing blood.[19] Image processing on a video stream can convert raw laser speckle images into color maps, where the color corresponds to the computed velocity. The physical mechanism of LSCI is similar to that of laser Doppler velocimetry; however, these systems are probe-based and provide measurements at a single point, whereas LSCI yields a velocity measurement at each pixel in a camera image.[20] It is hypothesized that LSCI has potential utility in laparoscopic urological surgery. Our objective was to investigate LSCI by comparing it with ICG in displaying renal perfusion changes in a porcine model, taking advantage of the unique imaging module form factor that allows the user to easily switch between modalities (Supplement, https://links.lww.com/CURRUROL/A25).
2. Materials and methods
We utilized a novel imaging module, ActivSight™ (Activ Surgical Inc., Boston, MA), which consists of an infrared-sensitive monochrome imaging sensor adapter and a customized light source with 1 or more coherent laser sources. In LSCI mode, proprietary algorithms detect perfusion using motion in real time, projecting a false color overlay on the standard white light laparoscopic image. This Food and Drug Administration–approved technology has the capacity for both ICG NIRF and LSCI. The imaging module was inserted between a standard laparoscopic system camera head and rigid laparoscope (Fig. 1A). Standard white light and coherent laser were combined with a specialized light guide attached to the module and laparoscope, respectively (Fig. 1B). In accordance with the Animal Welfare Act and hospital ethics, 2 anesthetized porcine models underwent laparotomy on 2 separate days by 2 different teams of surgeons: JN, CN, PK, YL, and SM. The bowel was mobilized to expose the retroperitoneum, with a focus on the left kidney, renal hilar, and great vessel anatomy. The laparoscope and adapter were mounted on a freehand stand that was aimed directly above the surgical field over the kidney. The laparoscopic video display was passed through the accompanying tower. The LSCI mode (ActivPerfusion™) of the adaptor displayed blood flow as a heatmap signature, where red and blue to no color indicated more perfusion and low to no perfusion, respectively. The NIRF mode (ActivICG™) displayed ICG dye concentration as a green intensity map. The investigational quantification mode displayed blood flow on a numerical scale.
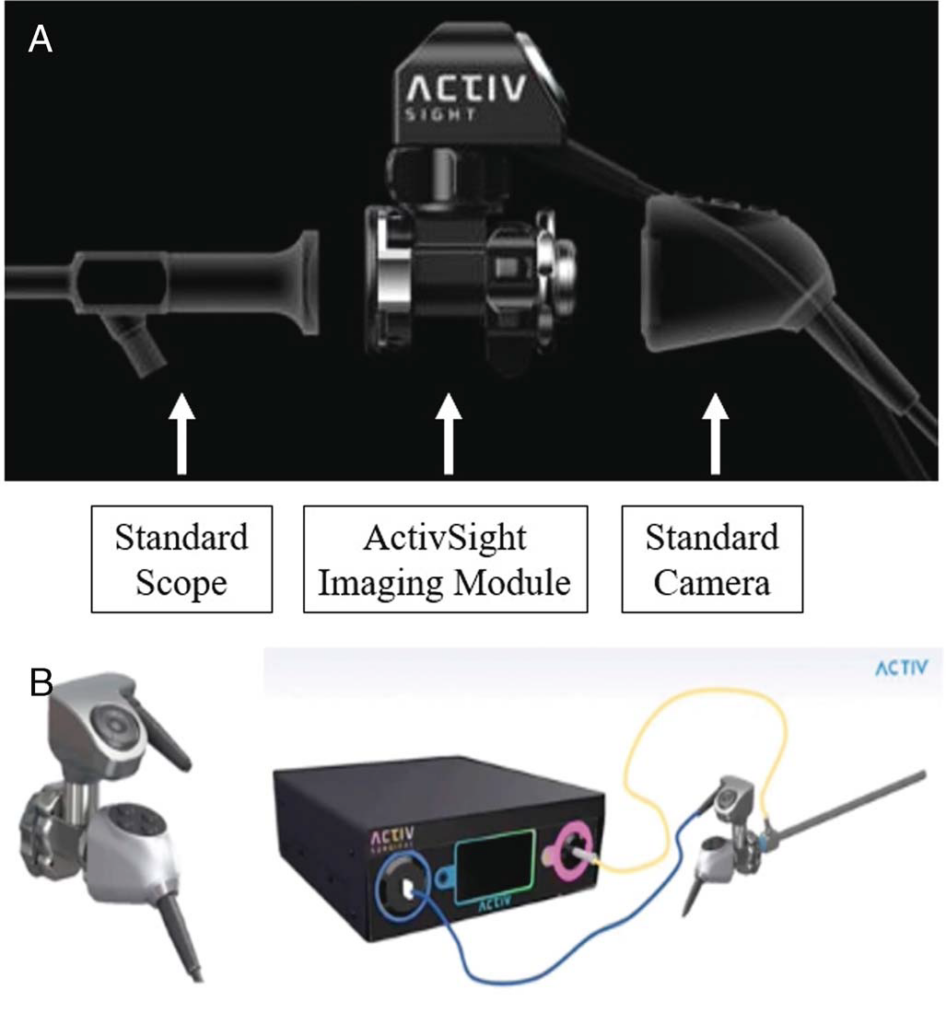
Indocyanine green (1.25 mg) was injected into the porcine model, and a control assessment of the extrahepatic biliary tree was performed to ensure green fluorescence. The left-sided upper and lower segmental renal arteries, renal vein, and aorta were isolated with rubber vessel loops, and a Cooley renal artery clamp was applied sequentially.
3. Results
Forty seconds after ICG administration with clamping of the upper segmental artery, ICG fluorescence was observed in the lower pole, with no fluorescence detected in the upper pole (Fig. 2A). When the renal parenchyma and hilar anatomy were visualized using LSCI (Fig. 2B), a red perfusion signal was noted in the lower pole with a perfusion boundary concordant with the boundary identified using ICG, whereas a low perfusion signal (blue color) was noted in the upper pole, corresponding to the lack of ICG fluorescence in the upper pole. After the upper segmental artery was unclamped, ICG was redistributed to the upper pole, as demonstrated by the increased fluorescence signal noted in the upper pole (Fig. 2C). In the LSCI mode, an immediate uniform red color observed over both poles of the kidney confirmed the restoration of the blood supply to the upper pole (Fig. 2D).
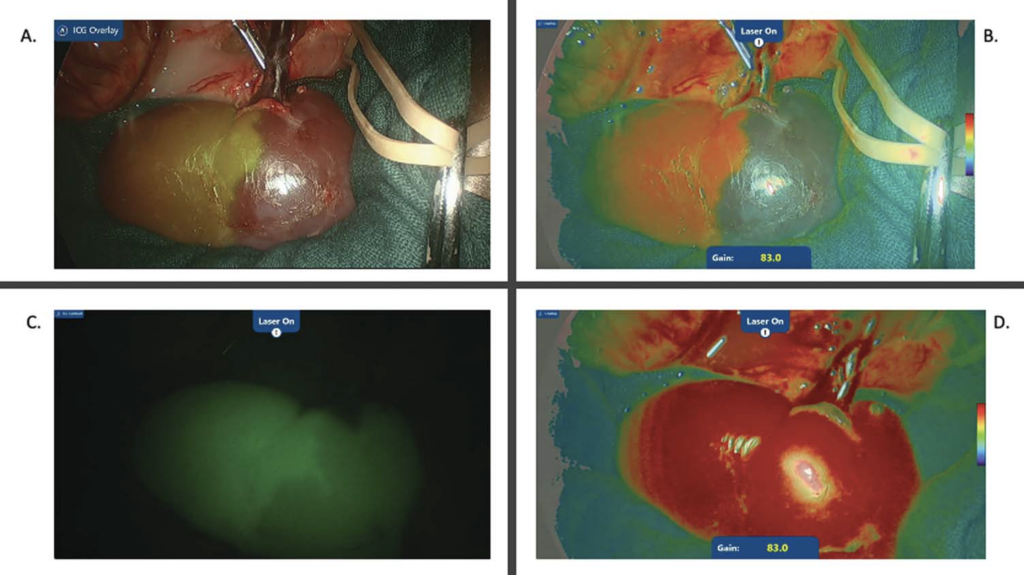
Figure 3A shows the left kidney after ICG administration and upper segmental artery clamping, with green demarcation of the lower pole using NIRF. As shown in Figure 3B, the investigational LSCI quantification mode demonstrated low quantification capture of the upper pole, reflected as 16.1 perfusion units. Figure 3C demonstrates an instant numerical increase in the upper pole LSCI units to perfusion 63.5, once the clamp was switched to the lower segmental renal artery. This was visually reflected by the change in the perfusion signal color compared with that in Figure 3B. When the clamps were removed from all arteries, the investigational LSCI quantification mode confirmed visually and numerically (52.1 perfusion units) that the blood supply had returned to the entire kidney (Fig. 3D). No intraoperative adverse effects were observed in any of the animals during the experiments.
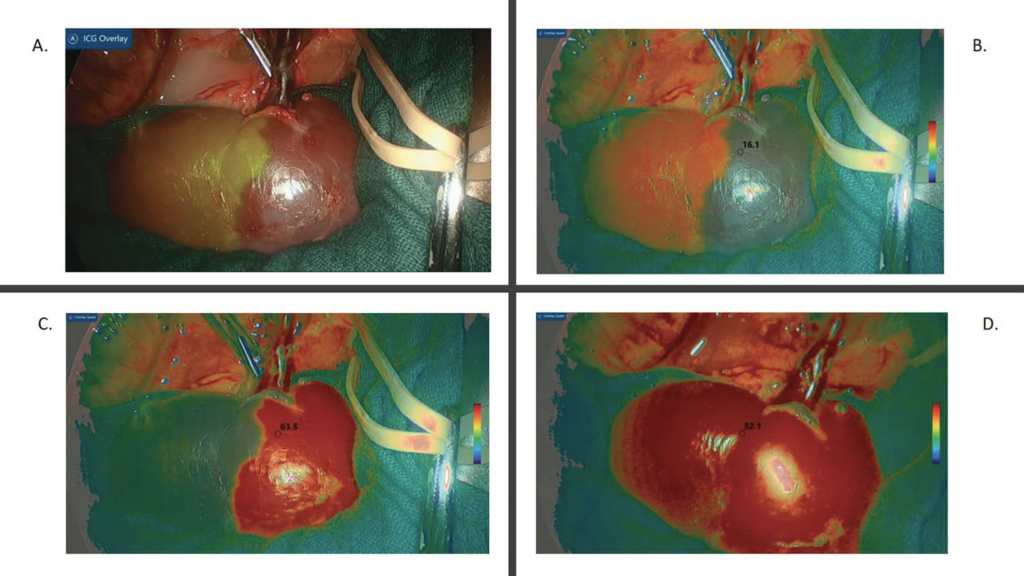
4. Discussion
We report the first use of the LSCI investigational quantification mode in a laparoscopic form factor for the real-time, dyeless, repeatable assessment of renal perfusion. Laser speckle contrast imaging perfusion signals demonstrated visual concordance with ICG for perfusion assessment of the kidney. In addition, the investigational LSCI quantification mode had higher perfusion units over perfused areas of the kidney and lower perfusion units over areas with limited perfusion. Our findings agree with those of other published findings that showed that the use of LSCI for perfusion assessment in the neural cortex and intestine was feasible.[21–23] Furthermore, LSCI has been assessed in research trials in cholecystectomy and colorectal and bariatric surgery settings (ClinicalTrials.gov identifier NCT04633512). A recent study that compared LSCI measurement of renal cortical microperfusion with total arterial renal blood flow and side-stream dark-field imaging demonstrated that LSCI was significantly correlated with both techniques. However, the correlation of renal blood flow with stream dark-field imaging was superior to that with LSCI in 1 study.[24]
Because LSCI is a dyeless visualization technology, it can be used in its different modes multiple times during the same procedure without any interference to its field of view. It also provides an objective relative quantification of flow and perfusion. In ICG-based visualization systems, dye retention in the tissue may affect the specificity of subsequent visualization of tissue, yielding various shades of green color that are subjectively interpreted by the user. This lends such systems false-positive results. Moreover, ICG-assisted visual systems do not provide quantification of blood flow or tissue perfusion.
Although LSCI avoids false-positive results from dye retention and allows for real-time measurement of tissue perfusion, it introduces a limitation in that ICG lacks motion artifacts. The laser speckle signal is generated by the motion of either tissue or blood. To accurately measure the blood flow, it is necessary for the target tissue to be relatively stationary. We observed that optimal use of the imaging module required adequate user stabilization during target organ analysis as motion tremor during adjustment of the LSCI image display resulted in motion artifacts in the order of milliseconds while switching among the different modes. Therefore, we hypothesize that motion stabilization or tremor elimination in robot-assisted laparoscopic surgery would further improve the utilization of this implementation of LSCI. In addition, algorithms may be used to minimize the motion sensitivity of LSCI technology.
The role of LSCI technology in urology is similar to that of the more widely used NIRF/ICG, where a focus on both laparoscopic and robotic renal surgery[25] exists for complex vascular anatomy cases. The clinical advantage of LSCI is the avoidance of drug administration along with a real-time adjunct for qualitative and quantitative renal parenchymal perfusion assessment. This approach seems reproducible and can hasten intraoperative decision-making on vascularity, particularly in PN. Digitally activated surgery will enhance augmented reality’s aim to impact perioperative outcomes.[26] A limitation of our study was the small sample size (n = 2). However, for the purposes of our study, it was necessary to use more than 2 porcine models to assess and demonstrate the feasibility of this new technology (LSCI) in detecting renal cortical microperfusion and comparing it with ICG.
Future work includes urology-led clinical trials using the dual-mode ActivSight Imaging Module, assessing its feasibility and safety evaluation during partial and radical nephrectomy, nerve-sparing prostatectomy utilizing the landmark artery,[27] and postoperative follow-up on their respective pentafectas.[28,29] Such trials should compare LSCI to standard white light with NIRF/ICG-enhanced laparoscopic and robotic surgery.
5. Conclusions
Laser speckle contrast imaging is a dye-free, repeatable, real-time adjunct for renal parenchymal perfusion assessment. Its applicability in minimally invasive renal surgery can complement the technology of ICG NIRF, improving visualization, and advancing digital surgery.
Acknowledgments
None.
Statement of ethics
Institutional Review Board approval to undertake this pre-clinical study was granted. Informed consent was obtained from all patients included in this study. All procedures followed were in accordance with the ethical standards of the responsible committee on human experimentation (institutional and national) and with the Helsinki Declaration of 1975, as revised in 2000.
Conflict of interest statement
No conflict of interest has been declared by the authors. VP is an advisor for Activ Surgical Inc. YL, VEB, JO, SM, AFD, and PCWK are employees of Activ Surgical.
Funding source
No funding was received funding for the preparation and work reported in this manuscript.
Author contributions
JN, PK, VP: Study concept and design;
AM, CAN, YL, VEB, JO, SM, AFD, PCWK: Data acquisition;
CAN, VEB, JO: Data analysis;
JN, AM, CAN: Drafting of the manuscript;
JN, CAN, VP: Critical revision of the manuscript.
References
1. Clayman RV, Kavoussi LR, Soper NJ, et al. Laparoscopic nephrectomy: Initial case report. J Urol 1991;146(2):278–282.
2. Leppert JT, Mittakanti HR, Thomas IC, et al. Contemporary use of partial nephrectomy: Are older patients with impaired kidney function being left behind? Urology 2017;100:65–71.
3. Mir MC, Derweesh I, Porpiglia F, Zargar H, Mottrie A, Autorino R. Partial nephrectomy versus radical nephrectomy for clinical T1b and T2 renal tumors: A systematic review and meta-analysis of comparative studies. Eur Urol 2017;71(4):606–617.
4. Huang R, Zhang C, Wang X, Hu H. Partial nephrectomy versus radical nephrectomy for clinical T2 or higher stage renal tumors: A systematic review and meta-analysis. Front Oncol 2021;11:680842.
5. Gadus L, Kocarek J, Chmelik F, Matejkova M, Heracek J. Robotic partial nephrectomy with indocyanine green fluorescence navigation. Contrast Media Mol Imaging 2020;2020:1287530.
6. Landsman ML, Kwant G, Mook GA, Zijlstra WG. Light-absorbing properties, stability, and spectral stabilization of indocyanine green. J Appl Physiol 1976;40(4):575–583.
7. Diana P, Buffi NM, Lughezzani G, et al. The role of intraoperative indocyanine green in robot-assisted partial nephrectomy: Results from a large, multi-institutional series. Eur Urol 2020;78(5):743–749.
8. Carty KN, Hwang A, Gordon A, et al. Indocyanine green (ICG) assessment of ureteral perfusion during pediatric robotic surgery. J Pediatr Surg Case Rep 2021;74:102058.
9. Kaplan-Marans E, Fulla J, Tomer N, Bilal K, Palese M. Indocyanine green (ICG) in urologic surgery. Urology 2019;132:10–17.
10. Petrut B, Bujoreanu CE, Porav Hodade D, et al. Indocyanine green use in urology. J BUON 2021;26(1):266–274.
11. Mangano MS, De Gobbi A, Beniamin F, Lamon C, Ciaccia M, Maccatrozzo L. Robot-assisted nerve-sparing radical prostatectomy using near-infrared fluorescence technology and indocyanine green: Initial experience. Urologia 2018;85(1):29–31.
12. Kumar A, Samavedi S, Bates A, et al. Use of intra-operative indocyanine green and Firefly® technology to visualize the “landmark artery” for nerve sparing robot assisted radical prostatectomy. Eur Urol Suppl 2015;14(2):eV36.
13. Abdelhafeez A, Talbot L, Murphy AJ, Davidoff AM. Indocyanine green–guided pediatric tumor resection: Approach, utility, and challenges. Front Pediatr 2021;9:689612.
14. Manny TB, Hemal AK. Fluorescence-enhanced robotic radical cystectomy using unconjugated indocyanine green for pelvic lymphangiography, tumor marking, and mesenteric angiography: The initial clinical experience. Urology 2014;83(4):824–829.
15. Gilshtein H, Yellinek S, Wexner SD. The evolving role of indocyanine green fluorescence in the treatment of low rectal cancer. Ann Laparosc Endosc Surg 2018;3(10):85.
16. Boni L, David G, Dionigi G, Rausei S, Cassinotti E, Fingerhut A. Indocyanine green–enhanced fluorescence to assess bowel perfusion during laparoscopic colorectal resection. Surg Endosc 2016;30(7):2736–2742.
17. Jafari MD, Wexner SD, Martz JE, et al. Perfusion assessment in laparoscopic left-sided/anterior resection (PILLAR II): A multi-institutional study. J Am Coll Surg 2015;220(1):82–92.e1.
18. van den Bos J, Wieringa FP, Bouvy ND, Stassen LPS. Optimizing the image of fluorescence cholangiography using ICG: A systematic review and ex vivo experiments. Surg Endosc 2018;32(12):4820–4832.
19. Draijer M, Hondebrink E, van Leeuwen T, Steenbergen W. Review of laser speckle contrast techniques for visualizing tissue perfusion. Lasers Med Sci 2009;24(4):639–651.
20. Briers JD. Laser Doppler, speckle and related techniques for blood perfusion mapping and imaging. Physiol Meas 2001;22(4):R35–R66.
21. Richards LM, Towle EL, Fox DJ Jr., Dunn AK. Intraoperative laser speckle contrast imaging with retrospective motion correction for quantitative assessment of cerebral blood flow. Neurophotonics 2014;1(1):015006.
22. Heeman W, Steenbergen W, van Dam G, Boerma EC. Clinical applications of laser speckle contrast imaging: A review. J Biomed Opt 2019;24(8):1–11.
23. Towle EL, Richards LM, Kazmi SM, Fox DJ, Dunn AK. Comparison of indocyanine green angiography and laser speckle contrast imaging for the assessment of vasculature perfusion. Neurosurgery 2012;71(5):1023–1030; discussion 1030–1031.
24. Heeman W, Maassen H, Calon J, et al. Real-time visualization of renal microperfusion using laser speckle contrast imaging. J Biomed Opt 2021;26(5):056004.
25. Tobis S, Knopf JK, Silvers C, et al. Robot-assisted and laparoscopic partial nephrectomy with near infrared fluorescence imaging. J Endourol 2012;26(7):797–802.
26. Vávra P, Roman J, Zonča P, et al. Recent development of augmented reality in surgery: A review. J Healthc Eng 2017;2017:4574172.
27. Patel VR, Schatloff O, Chauhan S, et al. The role of the prostatic vasculature as a landmark for nerve sparing during robot-assisted radical prostatectomy. Eur Urol 2012;61(3):571–576.
28. Sri D, Thakkar R, Patel HRH, et al. Robotic-assisted partial nephrectomy (RAPN) and standardization of outcome reporting: A prospective, observational study on reaching the “trifecta and pentafecta”. J Robot Surg 2021;15(4):571–577.
29. Patel VR, Sivaraman A, Coelho RF, et al. Pentafecta: A new concept for reporting outcomes of robot-assisted laparoscopic radical prostatectomy. Eur Urol 2011;59(5):702–707